Highlights of Aragats Research
А.Чилингарян; Физика высоких энергий в атмосфере Земли
(03.2021; ПРИРОДА - ЕЖЕМЕСЯЧНЫЙ ЕСТЕСТВЕННОНАУЧНЫЙ ЖУРНАЛ РОССИЙСКОЙ АКАДЕМИИ НАУК)
Национальная научная лаборатория имени А.Алиханяна (Ереванский физический институт) (Ереван, Армения)Новая методология физики высоких энергий в атмосфере характеризуется последовательным применением методов физики элементарных частиц и ядерной спектроскопии для выявления деталей работы ускорителей электронов, возникающих прямо над нашими головами в грозовых облаках. Совместно с потоками высокоэнергетических электронов и γ-лучей от лавины релятивистских убегающих электронов продолжительностью в несколько минут на исследовательской станции Арагац зарегистрированы также часовые изотропные потоки низкоэнергетических γ-лучей от изотопов 222Rn. Каждый вид космических лучей приносит свои особые признаки, позволяющие оценить структуру и силу атмосферного электрического поля.Эффект торможения мюонов, наблюдаемый одновременно с крупнейшим зарегистрированным увеличением потока электронов и γ-лучей на гореЛомницкий Штит, позволяет оценить максимальное значение атмосферного электрического поля. Измеряя энергетические спектры естественногоγ-излучения, мы обнаружили новый эффект циркуляции дочерних продуктов радона во время гроз. Сравнение энергетических спектров электронов и γ-лучей грозовых наземных превышений позволяет исследовать возникающие электрические структуры в атмосфере, которые ускоряют затравочные электроны космических лучей до ≈70 МэВ. Измеряя одновременно потоки нейтронов и γ-лучей нейтронным монитором и гибриднымдетектором частиц SEVAN, мы смогли доказать фотоядерное происхождение атмосферных нейтронов...читать далее
INTRODUCTION
Aragats research station (Figs. 1,3) of the Artem Alikhanyan National lab (Yerevan Physics Institute) with its unique equipment for measuring particle fluxes and atmospheric discharges is located on Mt. Aragats, at a distance of about 50 km from Yerevan. Aragats is an inactive, cone-shaped volcano with a diameter of about 40 km at its base. Its slopes are characterized by radial valleys that are deeply carved into the permeable volcanic rock. One of the world-first and largest high-mountain research stations was established on Mt. Aragats, at 3200 m elevation, 77 years ago in the middle of World War II in 1943. Since then, expeditions on Aragats continued uninterruptedly, in spite of insufficient funding, and electricity and fuel shortages during the recent history of Armenia. Currently, physicists of the Cosmic Ray Division of Yerevan Physics Institute with a reequipped and renewed facilities continue research in the field of galactic and solar cosmic rays, solar-terrestrial connections, atmospheric physics, and space weather. The main topic of research previously was the physics of the high-energy cosmic rays accelerated in our Galaxy and beyond (see review and references for early Aragats research in Chilingarian et al.,2009).
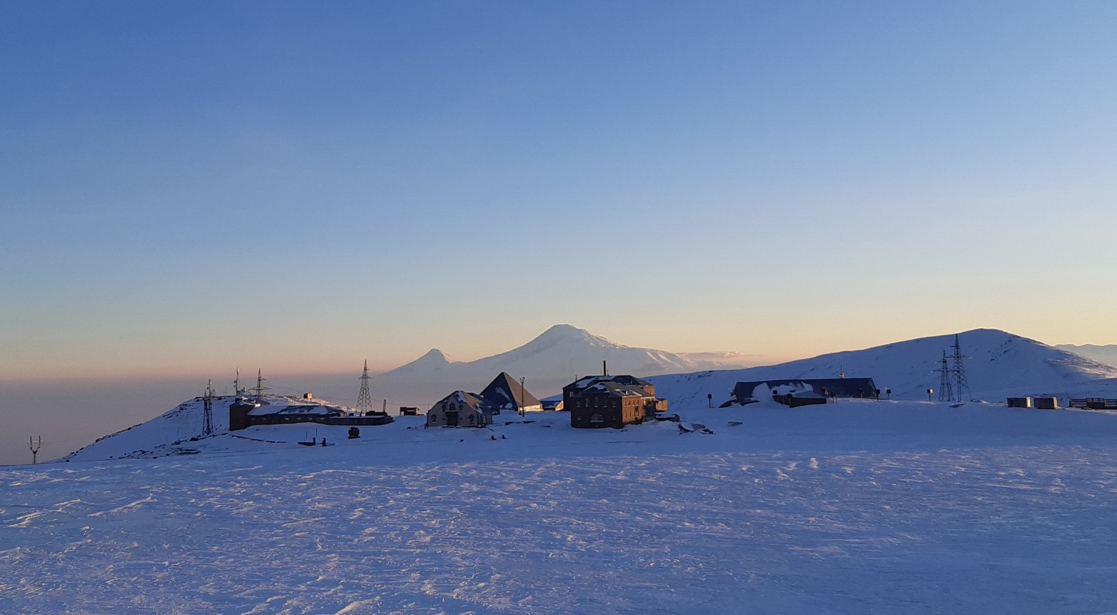
Two surface arrays consisting of hundreds of plastic scintillators were measuring extensive air showers (EASs), the gigantic cascades of particles born in interactions of the primary high-energy proton or fully stripped nuclei with atoms of the terrestrial atmosphere. Since 2000, the CRD has been conducting constant monitoring of the cosmic ray flux at Aragats to study proton accelerators on the Sun and to alert about the dangerous consequences of solar flares. Fortunately, since the 90s, we have left a lot of elementary particle detectors from large ground-based installations that register extensive air showers (EAS), particle fluxes from interactions of protons and nuclei, accelerated to ultrahigh energies by galactic and extragalactic accelerators. After the completion of the EAS experiments on Aragats, research began on a new interesting topic - solar physics and space weather. Neutron monitors located at an altitude of 3200 and 2000 m, and a multitude of new particle detectors measuring the charged and neutral components of secondary cosmic rays, make Aragats one of the largest centers for the study of solar-terrestrial communications. During the 23rd solar cycle, many important solar energy events were measured, including the largest series of ground-level enhancements (GLE) and Forbush decreases in November 2003 (the so-called Halloween events, Chilingarian & Bostanjyan, 2010, see Fig. 2), and huge fluxes of the high-energy solar protons (Bostanjyan and Chilingarian, 2007, Chilingarian, 2009). As we can see in Fig. 2 ground level enhancements (GLE) and Forbush decreases (FD) are well pronounced at high latitudes (Oulu neutron monitor) comparing with middle and low altitudes (Aragats) due to in the first case registration of the huge amount of low energy secondaries from the solar protons, and in the second case, visa-verse suppression of the low energy cosmic ray flux. However, the geomagnetic storm (GMS) is better pronounced on the middle-low latitudes because due to lowering of the cutoff rigidity a huge amount of low energy secondaries from the galactic cosmic rays usually bended from Aragats location by the geomagnetic field.
Figure 2. Halloween events of 2003. By different colors we demonstrate galactic cosmic ray modulation effects by the violent solar activity (solar flares, interplanetary coronal mass ejections and shock waves).
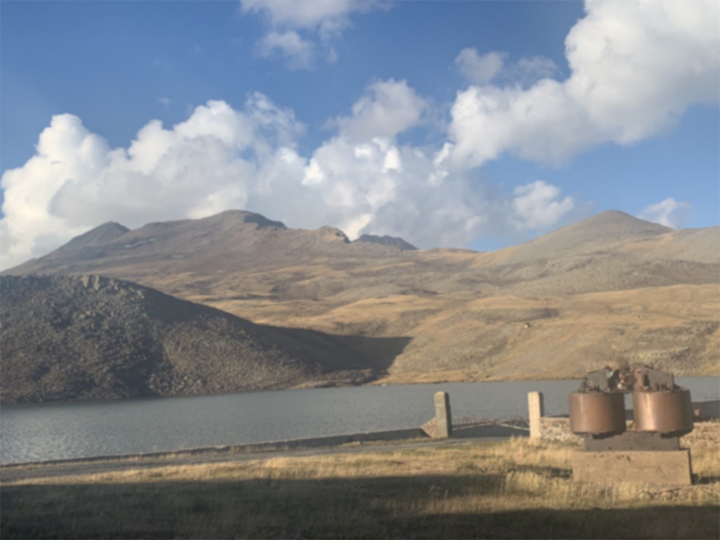
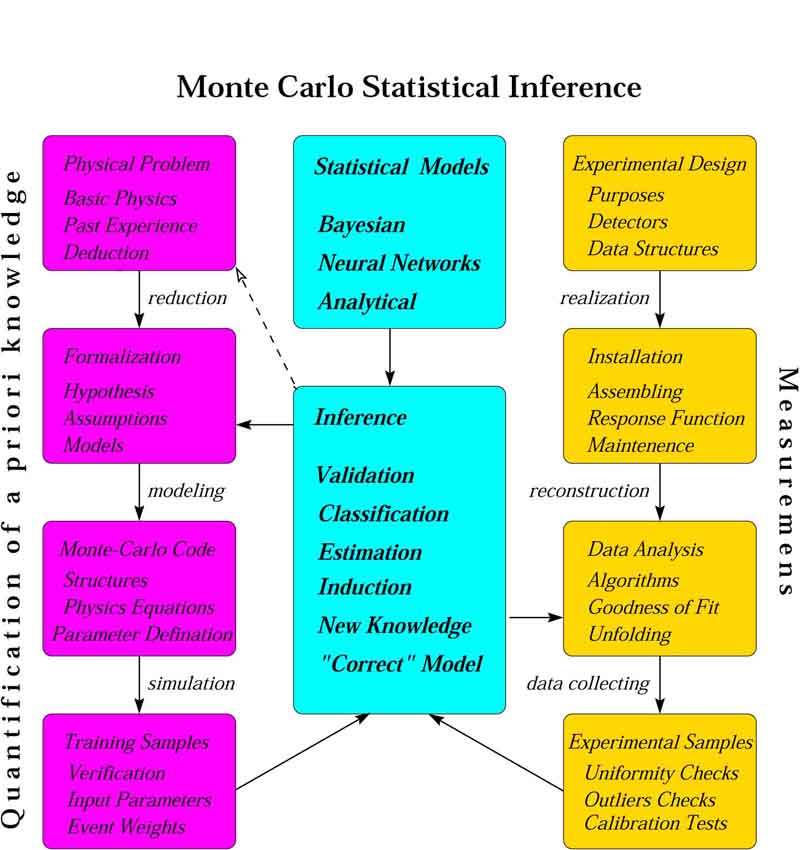
More than a million EASs detected in 1999-2004 have been carefully examined and used for the estimation of energy spectra of light and heavy nuclei (Chilingarian et al, 2004). In Fig. 5b we show the energy spectra of light and heavy nuclei obtained by the methodology shown in Fig. 5a. The evidence from the recovered energy spectra can be summarized as follows (Vardanyan et al., 1989, Chilingarian et al., 1999):
- The estimated energy spectrum of the light mass group of nuclei shows a very sharp knee: Dg ≈ 0.9, compared to ≈ 0.3 for the all-particle energy spectra.
- In contrast, the energy spectrum of the heavy mass group of cosmic rays shows no break in the energy interval of 1015 - 2x1016 eV.
- The MAKET-ANI results on the rigidity-dependent position of the knee, points towards SuperNovae remnants (SNRs) as a most favorable source of galactic cosmic rays and Fermi-type acceleration as the mechanism of hadron acceleration. Further results from the KASCADE array and the AGILE and Fermi orbital gamma ray observatories, as well as several theoretical extensions of Fermi mechanism, confirms MAKET-ANI results.
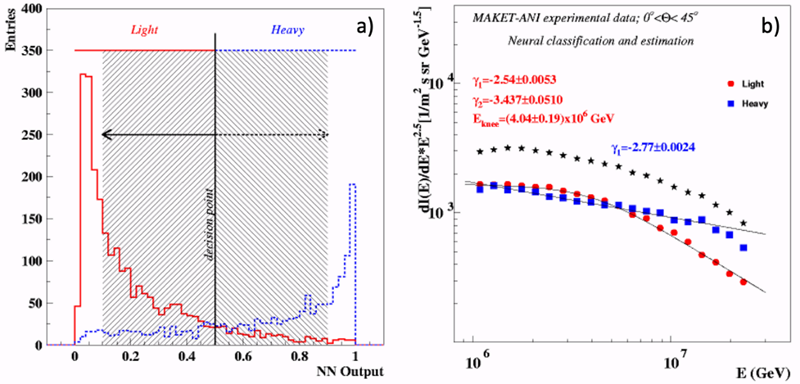
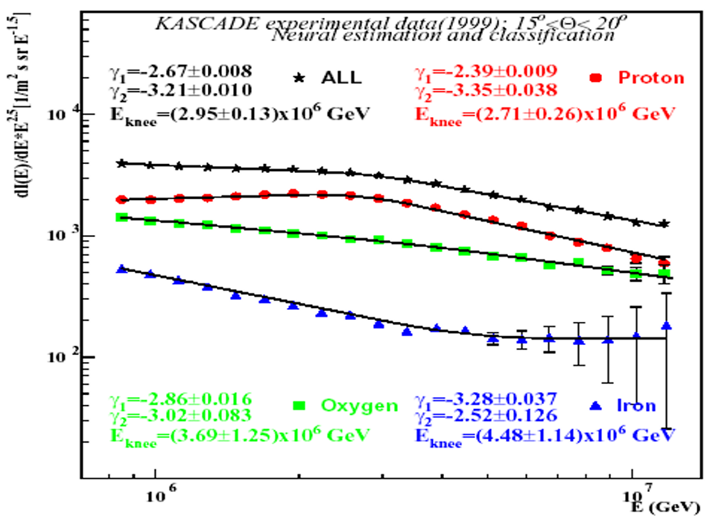
|
MAKET | KASCADE |
light heavy | light heavy | |
light heavy | 0.720 0.280 0.240 0.760 | 0.688 0.312 0.338 0.662 |
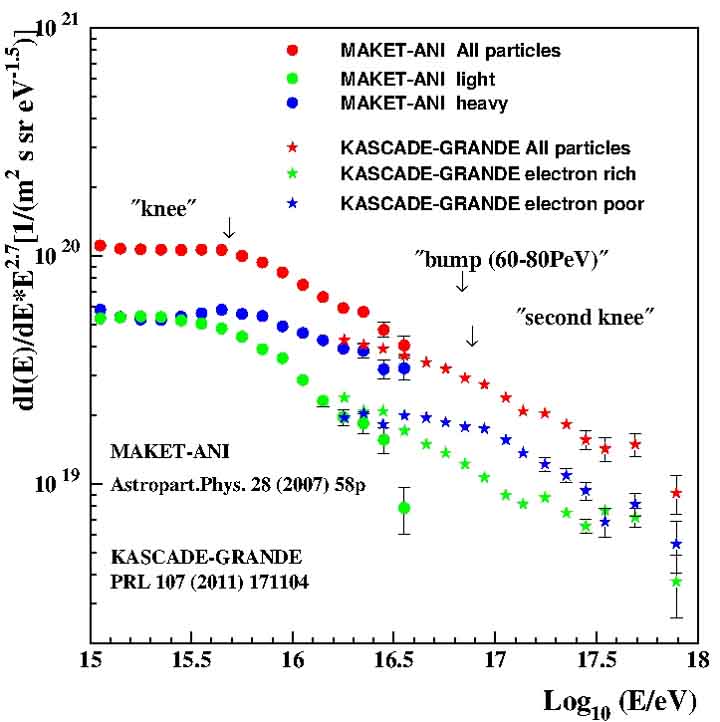
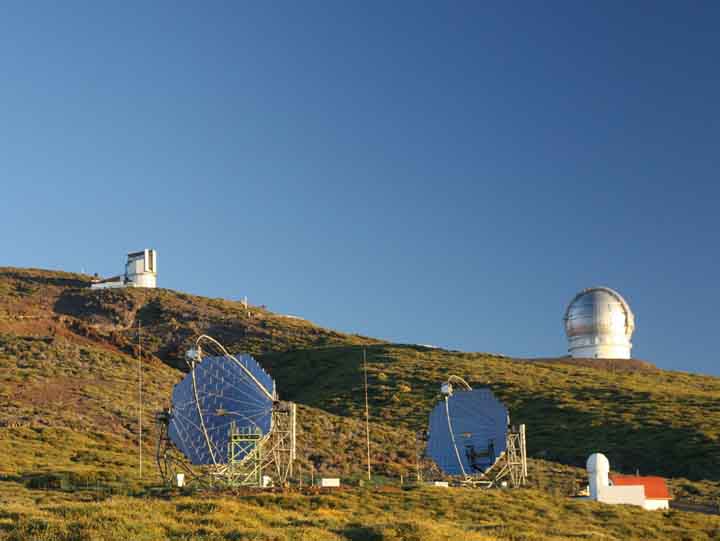
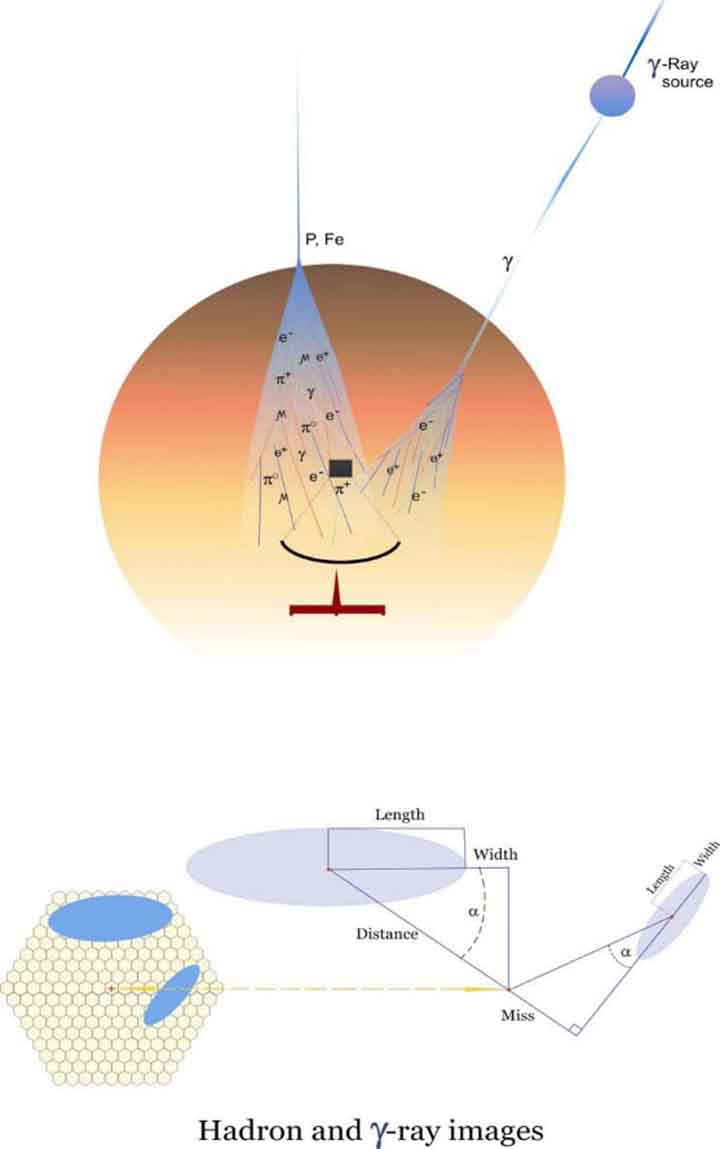
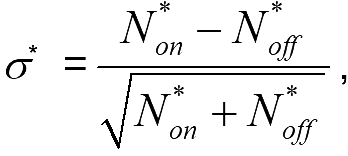
|
N*on | N*off | s | DIFF | DIFF/N*off | N*off/ Noff |
Raw
Azwidth Wedgecut1 Supercut 2 Neural3 4::5::1 |
506255 14622 6017 4452 6278 |
501408 11389 3381 1766 2858 |
4,8 20.4 27.2 34.3 35.8 |
4847 3233 2636 2686 3420 |
0.01 0.28 0.78 1.52 1.20 |
0.0227 0.0067 0.0035 0.0057 |
2Punch, M., C.W. Akerlof, M.F. Cawley, et. al. Supercuts: an improved method of selecting gamma-rays. Proc. 22nd Internal. Cosmic Ray Conf., Dublin, 1, 464-467, 1991
However, the question remains how to find an optimal set of parameters to reject the background and not too strict suppress the efficiency of event selection. Physicists from CRD solve this problem by introducing a new method directly optimizing the nonlinear multidimensional shape of the best gamma-cluster (Chilingarian, 1994, Chilingarian and Cawley, 1994, Chilingarian, 1995), Fig.11. In this method, we do not use the a-priory information on the shape of the signal domain. This shape is very difficult to simulate due to a variety of very high-energy gamma ray sources and numerous random and methodical errors. Using only experimental information from the scans when telescope axes were pointed on the source in question and open space, we avoid additional uncertainties connected with using nonreliable a-priory information. Thus, we use only experimental information: the source + background and pure background. In the upper row of Fig. 10, we show the model of signal (nonlinear shape) overlaid on the background and uniformly distributed random background. In the middle row we show one of the steps of working of iterative algorithm optimizing the signal domain shape, and in the bottom row - the final shape that covers signal domain and minimally includes background. 2 algorithms were used (left and right sides of Fig. 10, see details in Chilingarian, 1997).
Figure 10. Implementation on a toy problem the new type of NN - Mapping Networks (MP). MP maximizes the signal significance, directly optimizing the shape of the Gamma Cluster.
T. Antoni, W.D. Apel, A. Chilingarian, et al., KASCADE Measurements of Elemental Groups of Cosmic Rays: Results and Open Problems, Astroparticle Physics, 24, (1-25), 2005.
Chilingarian, A.A., Classification of the gamma and proton images with aid of mathematical models of Nural Networks, Proc. 22ICRC, 1, 483, Dublin, 1991b.